Team:Imperial College/Industrial Implementation
From 2013.igem.org
Contents |
Industrial Implementation
Summary
Mixed waste is particularly difficult to manage due to limitations in current technologies. We have identified solid recovered fuel (SRF), mainly composed of plastics and paper that cannot be recycled in existing recycling facilities, as our market niche. The objective of our technology is to build on current recycling infrastructure and make use of resourceful waste to produce bioplastics. Since the SRF, the feedstock of our engineered bacteria, is a burden to recycling companies, we can make profits through selling our products poly-3-hydroxybutyrate (P3HB) and ethylene glycol and charging the companies for sending SRF to us.
Product details
Using mixed waste as a resource, our system has been designed to produce poly-3-hydroxybutyrate and ethylene glycol as commodities to be sold in industry.
P3HB is industrially biodegradable, breaking down without release of toxic products or intermediates. It has a low water solubility [1] and medium-low water and CO2 permeability [2], as well as very good barrier properties that are ideal for packaging. This biopolymer also has a high degree of crystallinity, high melting temperature [3], and good tensile strength, making it comparable to one of the most common petroplastics; isotactic polypropylene [3]. In addition, there are many processing methods for P3HB [4], such as injection molding and extrusion, which are required in manufacture of most plastic products. Although the impact strength is relatively weaker compared to petroplastics, P3HB nanocomposites have shown significantly improved impact strength as well as other properties [3].
Ethylene glycol is a valuable by-product obtained in the process of recycling PUR and PET. Due to the high affinity for water, ethylene glycol has been commercialised for a wide range of uses including desiccant and vaccine manufacture.
Overview
Sourcing
Our waste degradation system is designed to enhance current waste management practices, particularly in managing mixed waste. One type of mixed waste, called solid recovered fuel (SRF), is produced from recycling plant seeking to recover valuable products from waste. In just the one mixed recovery facility we visited alone, about 320,000 tonnes of SRF, 20% of which is plastic, is produced per year. It is then shipped to other countries for incineration. SRF is a cost to these recycling companies as they pay approximately £65 per tonne for shipping. Our plan is to use SRF from the recycling companies as the feedstock, charging less than incineration costs but still being payed a fee. From SRF we will extract the product ethylene glycol and produce a substrate which we can produce the commodity bioplastic, P(3HB) from.We have also designed the first system for P(3HB) recycling, taking responsibility for the end of life solutions to the commodity we are producing.
Siting
We plan to operate the bioreactors for SRF breakdown at the mixed recovery facilities(MRFs) which produce it originally. The purpose of these facilities is to recover anything of value from waste. At the MRF we visited, Powerday, we were told that a system which could efficiently produce valuable commodities from unrecoverable waste would be welcomed. One of the most important factors for economic viability is a ready access to the substrate, in this respect the MRF is an ideal location.
Consulting Industry
We contacted one of the leading P(3HB) producing companies in the world, [http://www.metabolix.com/ Metabolix], to discuss the important considerations of industrialisation. This is an excerpt from our conversation:
This is based on covering capital and other costs at a production level of 50,000 tons of P(3HB) a year. To produce 300,000 tons of glucose we have estimated a requirement of 781,250 tonnes of SRF a year. This is based on cellulose to glucose conversion rates and composition of SRF(see below). This is a reasonable amount with 320,000 tonnes of SRF produced at Powerday per year alone.
Utilising Cellulose Degradation
Many of the materials in SRF are cellulosic. Cellulose contains sugars in an inaccessible form for the the bacteria we are using. In order to maximise the efficiency at which we use SRF we need to release these sugars. Hydrolysis of cellulose using cellulases is sufficiently developed that we can utilise this in our system as they already do in fuel ethanol production. Below are our workings showing an estimated efficiency for P(3HB) production from SRF we might expect to get with out current system coupled with hydrolysis of cellulose.
We estimate 2.11% conversion of SRF to PHB by mass
80% SRF is paper, wood and fibre. 60% Efficiency of cellulose extraction. Our current bacteria convert glucose to P(3HB) with a 5.5% efficiency.
80% x 80% x 60% x 5.5% = 2.11%
Measurements of the mass of the constituents of SRF revealed that wood, paper and fibers are 80% by mass. (There will be some variation since the processes which produce the SRF take in different commercial waste every day) We have measured the weight of the two representative piles of separated SRF and calculated the % distribution. (W/P/F: 97.21g, Plastic: 19.48g)
We estimate that about 80% of these materials is cellulose and hemicellulose. This is the component of the equation which is likely to vary the most since it depends on the composition of waste that is processed by the mixed recycling facility. Paper is 100% cellulose. Fibres and textiles can be 100% cellulose (cotton) but can be blended with plastic or rubber. We estimate about 80% of fibres is cellulose. In wood, the cellulose and hemicellulose content is about 70% [8]. It has been recently demonstrated that hemicellulose can also be used for PHA production [9].
We estimate that 60% of cellulose can be converted to glucose by currently available technologies. The toughest cellulosic substrates are crystalline insoluble forms like paper. Some of the latest methods can achieve as high as 74.4% conversion even with these substrates [10]. Therefore, we are confident that it is possible to efficiently convert 75% of cellulose from wood-paper-fibres into glucose. A recent review [11] confirms that chemical and ennzymatic cellulose hydrolysis techniques are very advanced and efficient. It is possible to upscale cellulolyc technologies to industrial levels [12] . However, we estimate that this will reduce the efficiency from the 75% in the lab to about 60% in industry.
We can convert 5.5% of glucose into PHB with our hybrid operon in E.coli. In our experimental results, we produced 1.66 g/L P(3HB) on 3% glucose LB media, grown for 1 day. Therefore, we calculate that 8% (2.48/30=0.083) of the glucose is converted with this method. This efficiency could be increased by optimizing growth conditions and further perfected via metabolic engineering of the E.coli chassis.
Our models predicted similar conversion from glucose to PHB. In our metabolic model, when glucose is used as the only input to produce P(3HB). The extracellular glucose concentration is 200mM, which is approximately the concentration in 3% LB media.The modelling result showed a 1.77 g/L P(3HB) concentration after all the glucose was used up by the cells.
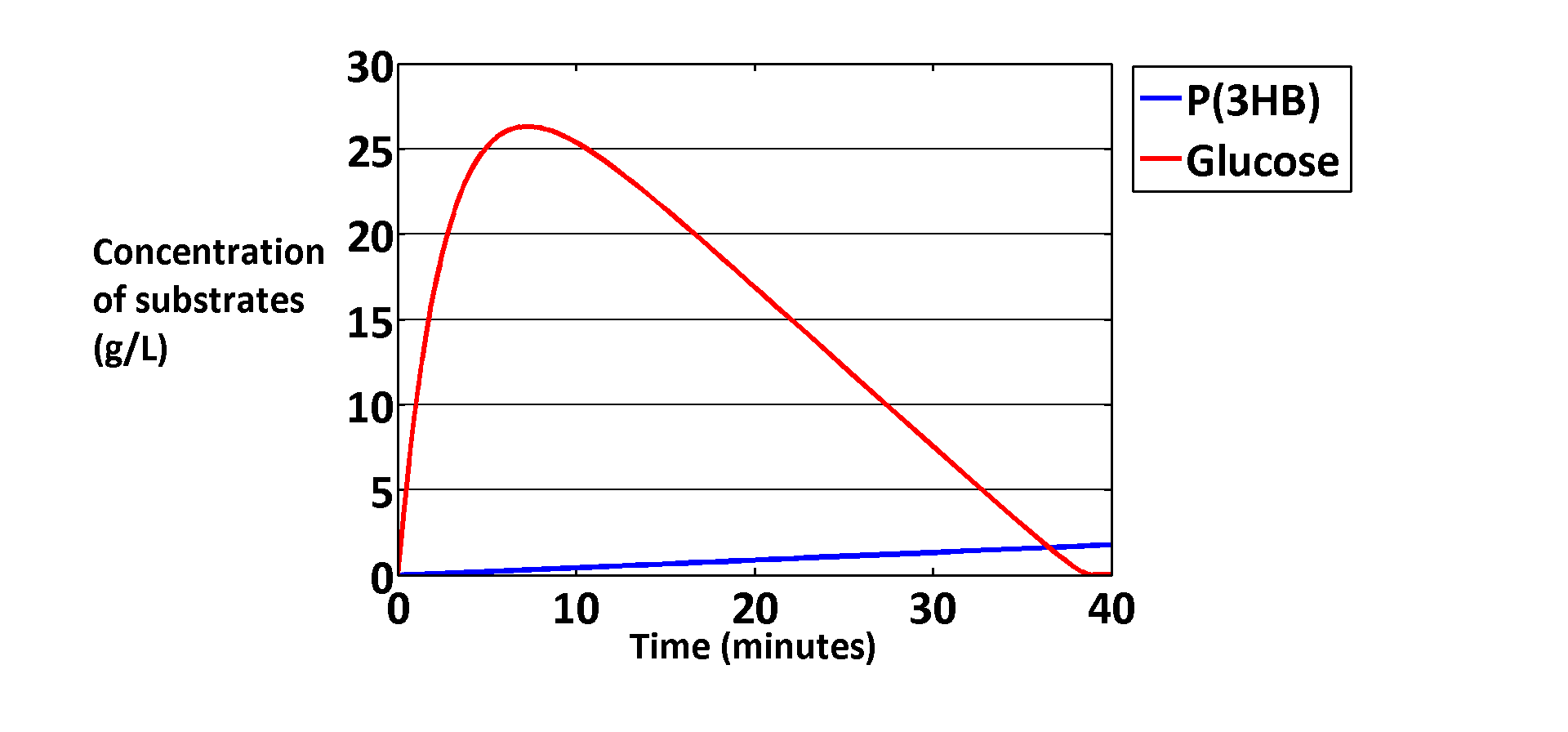
Here is our model used for industrial implementation. The details about the metabolic model are described in module 2. The model has only one input which is the extracellular glucose at the upper left corner.

Market analysis
Market for bioplastics
Plastics represent a large percentage of materials used, as they can be applied in products ranging from water bottles and EE (electrical and electronic) equipments to agricultural mulch film and textiles.
Although bioplastics count only a small percentage of the total plastics currently, driven by the increasing petroleum price, consumer and policy pressure of using greener products, the market for bioplastics is predicted to grow 30% per year [3], and market share is predicted to approach 25%-30% by 2020 [5]. Among all types of bioplastics, biopolyesters such as PHB are predicted as the drive for the market [7]. This significant increase in market share also imply that our bioplastics recycling technology has great importance in the near future, as it contributes to building a circular economy encouraged by the legislations.
Competitive comparison
We have carried out a rough analysis, comparing the potential cost of P(3HB) made using our system to that made from plant biomass[13]. Replacing the cost of the biomass with that of the SRF produces P(3HB) with a lower cost. The figure may not be entirely accurate due to the difficulties in estimating costs for such a complex system but it can be used as an indicator for the viability of using this 'advantaged' feedstock(in terms of cost). There are existing P3HB producing companies that utilise genetically modified plants or microbes to produce P3HB. Plant-based technology cost estimation is based on switchgrass, which shows the highest P3HB production efficiency (3.7% of total dry mass) compared to other plants [6]. The fact that our system will not compete for land, water and crop resources is one of the major benefits of our system over other microbial P(3HB) production.
References
- Terada, M. & Marchessault, R. H. (1999) Determination of solubility parameters for poly(3-hydroxyalkanoates). International Journal of Biological Macromolecules. . 25 (1-3), 207-215.
- Miguel, O., FernandezBerridi, M. J. & Iruin, J. J. (1997) Survey on transport properties of liquids, vapors, and gases in biodegradable poly(3-hydroxybutyrate) (PHB).Journal of Applied Polymer Science.. . 64 (9), 1849-1859.
- Pilla, S. (2011) Handbook of Bioplastics and Biocomposites Engineering Applications 1st edition. USA, Scrivener Publishing LLC.
- EHuang, J. C., Shetty, A. S. & Wang, M. S. (2003) Biodegradable plastics: A review. Advances in Polymer Technology. 10 (1), 23-30.
- Helmut Kaiser consultancy. (2010) Bioplastics market worldwide 2010/11-2015-2020-2025. [Online] Hong Kong. Available from http://www.hkc22.com/bioplastics.html. [Accessed: June 2013].
- Somleva, M. N., Snell, K. D., Beaulieu, J. J., Peoples, O. P., Garrison, B. R. & Patterson, N. A. (2008) Production of polyhydroxybutyrate in switchgrass, a value-added co-product in an important lignocellulosic biomass crop. Plant Biotechnology Journal.6 (7), 663-678.
- Research and Markets. (2012) Bioplastics - A Global Market Watch, 2011 - 2016. [Online] U.S. Available from http://www.researchandmarkets.com/research/z7dzsv/bioplastics_a
- Chemical Composition of Wood. Available from http://download.springer.com/static/pdf/51/art%253A10.1007%252Fs10295-006-0131-2.pdf?auth66=1382696953_3c5ff2886715fb5ef42a34ca0810f000&ext=.pdf
- Available from http://download.springer.com/static/pdf/51/art%253A10.1007%252Fs10295-006-0131-2.pdf?auth66=1382696953_3c5ff2886715fb5ef42a34ca0810f000&ext=.pdf
- Available from: http://www.ncbi.nlm.nih.gov/pmc/articles/PMC2849193/
- Available from: http://onlinelibrary.wiley.com/doi/10.1002/biot.201200033/pdf
- http://www.novozymes.tv/video/8756366/a-new-era-begins
- http://www.sciencedirect.com.iclibezp1.cc.ic.ac.uk/science/article/pii/S0263876298716582?np=y